Passage dependence of NADH redox status and reactive oxygen species level in vitro in triple-negative breast cancer cell lines with different invasiveness
Highlight box
Key findings
• The correlation between reduced nicotinamide adenine dinucleotide (NADH) redox status, reactive oxygen species (ROS) levels, and invasiveness in studied triple-negative breast cancer (TNBC) cells is not very sensitive to the passage number of TNBC cultures.
• The more invasive MDA-MB-231 cells exhibit more oxidized NADH redox status and higher cytoplasmic ROS levels than the less invasive HCC1806 cells under both basal condition and metabolic perturbations.
What is known and what is new?
• More oxidized NADH redox status detected by optical redox imaging and higher ROS levels have been associated with breast cancer aggressiveness in vitro and ex vivo. However, the cell cultures have phenotypic drift, thus redox metabolism may change with the passage number. Here we reported data on the passage-dependence of NADH redox status, plasticity, and ROS levels in two TNBC cell lines (one is more invasive than the other).
• The change of NADH redox status can be discordant with the change of ROS levels in breast cancer cells. Here we confirmed this for cytoplasmic and mitochondrial ROS levels in two TNBC cell lines.
What is the implication, and what should change now?
• The redox metabolism and its association with the invasive/metastatic potential of TNBC cells are not very sensitive to the passage number.
• NADH redox and cytoplasmic ROS status in TNBC likely reflect the intrinsic progressive nature of TNBC cells. They should be further investigated as potential prognostic biomarkers.
Introduction
Background
The redox status of nicotinamide adenine dinucleotide (NAD; including oxidized form NAD+ and reduced form NADH) is a key factor mediating various biological processes (1,2). The redox potential, NAD+/NADH, is coupled with a number of metabolic enzymes and mediates glycolysis, the tricarboxylic acid (TCA) cycle and respiration activities, as well as reactive oxygen species (ROS) generation. Optical redox imaging (ORI or called optical metabolic imaging sometimes in the literature) can detect NADH redox status based on the intrinsic fluorescence from NADH and oxidized flavoproteins (Fp) containing flavin adenine dinucleotide (FAD) (3). Optical redox ratio (ORR), i.e., Fp/(NADH + Fp) or FAD/(NADH + FAD), has been shown to correlate with NAD+/(NADH + NAD+) (4,5) and bioenergetics activities (6,7). ORI can be implemented with the Chance redox scanning (3,8) and single photon or multiphoton fluorescence microscopy, and has been widely used in the recent decades, often in combination with fluorescence life time imaging, for probing disease pathology and developing diagnostic and treatment strategies (9). ORI has been increasingly used to understand the role of NADH redox status in cancer transformation and progression and to develop imaging biomarkers for diagnosis and monitoring treatment response. ORI-detected NADH redox status was found to respond to the overexpression (5) and knockdown (10,11) of oncogenes, suggesting the interplay of genetic pathway and redox metabolism. Furthermore, more oxidized redox status with higher ORR was correlated with cancer transformation and the increase of cancer invasive/metastatic potential (12-16), implicating the potential of ORR as a prognostic biomarker. ORI has also successfully detected treatment response in various cancer models (3,17-22).
ROS have been implicated as key mediators in cancer development and progression, and high ROS level is commonly interpreted as higher oxidative stress associated with a more oxidized redox state. Recently, we investigated the relationship between NADH redox status and associated ROS release in triple-negative breast cancer (TNBC) cell cultures (23). The key finding was that both oxidized and reduced NADH redox status can correlate with ROS generation, and the relationship between NADH redox status and ROS levels may vary depending on cell lines.
Rationale and knowledge gap
It is well known cancer cells may experience phenotypic drifts in, e.g., morphology, growth rate, gene and protein expression, and response to treatment after repeated culturing in vitro (24-30). The extent of drift or variations are both cell line specific and propagation passage number dependent. Metabolic/redox reprogramming is recognized as a general feature of cancer progression. However, there are few studies on whether and how the metabolic/redox features of cancer cells change with passage number in vitro. Such information will be valuable for understanding cancer metabolism and development of metabolic biomarkers through in vitro studies. If the potential metabolic biomarkers drift significantly with the passage number, this will raise questions regarding the validity of studies identifying biomarker candidates by comparing metabolic characteristics of cell lines in vitro, since the passage number should then be under strict control.
Objective
To further confirm the relationship between NADH redox status, ROS level, and cancer aggressiveness, in this study we characterized the dependence of NADH redox and ROS profiles on the passage number for two TNBC cell cultures, i.e., MDA-MB-231 and HCC1806, which belong to different TNBC molecular subgroups (31) and differ in progression aggressiveness (invasive potential) (32,33). MDA-MB-231 belongs to mesenchymal stem-like subtype and HCC1806 belongs to basal-like subtype 2. MDA-MB-231 cells are more invasive than HCC1806 cells in vitro according to the invasion assay. We evaluated whether late passage TNBC cells experience drift in their redox and ROS profiles when compared to early passage cells, and how the NADH redox/ROS profiles are associated with cancer cell invasiveness under different passages and metabolic perturbation conditions. We will test the hypothesis that the redox/ROS profiles of TNBC are not sensitive to passage numbers, reflecting the intrinsic progression nature. We present this article in accordance with the MDAR reporting checklist (available at https://tbcr.amegroups.com/article/view/10.21037/tbcr-24-36/rc).
Methods
Cell culture
TNBC cells, HCC1806, and MDA-MB-231 were obtained from the American Type Culture Collection (ATCC). They were originally isolated from breast cancer patients, with the HCC1806 from the primary tumor of a 60-year-old Black female with negative lymph node status and MDA-MB-231 from the pleural effusion of a 51-year-old White female with treatment resistance and poor prognosis (https://www.atcc.org) (34). Both cell lines were maintained in T-25 flasks with Rosewell Park Memorial Institute 1640 medium (Gibco, RPMI 1640; Thermo Fisher Scientific, Waltham, MA, USA; Cat #11875085) supplemented with 10% fetal bovine serum (FBS). Cells were incubated at 37 ℃ with 5% CO2 and passaged using 0.25% trypsin-ethylenediaminetetraacetic acid (EDTA) at ~80% confluency. Early passage cells in this study are arbitrarily defined to be passaged less than 20 times. Late passage cells are defined to be passaged more than 60 times.
Live cell ORI
Cells were seeded 80,000/mL or 50,000/mL and grown for 1 or 2 days, respectively, in 20 mm glass-bottom well in 35 mm dishes (Cellvis, Mountain View, CA, USA; Cat #D35-20-1.5-N). One hour before imaging, cells were rinsed twice with Dulbecco’s phosphate buffered serum (DPBS+; with calcium and magnesium) and incubated with 1 mL live cell imaging solution (LCIS+; Molecular Probes, Eugene, OR, USA; supplemented with 11.1 mM glucose and 2.0 mM glutamine). To image we used a Zeiss wide field microscope (Axio Observer 7, Colibri 7 LED light source, Zeiss Axiocam 702 monochrome CMOS camera, and software ZEN Blue 2.5.75.6) with a 20× lens (numerical aperture 0.8) with the chamber environment set at 37 ℃. Fluorescence signals were collected through optical bandpass filters as follows: NADH excitation (Ex) 370–400 nm, emission (Em) 414–450 nm, exposure time 3 s; Fp Ex 450–488 nm, Em 500–530 nm, exposure time 2 s. Image matrix size is 1,920×1,216 with a pixel resolution of 0.293×0.293 µm2. Transmitting light was used to find and focus on regions of interest and generate bright field images. We imaged 3–5 randomly selected, distinct fields of view per dish as technical replicates. For each cell group, we conducted experiments on 2 separate days with total imaging dishes n=6 as biological replicates. All redox imaging experiments were accomplished using the same instrument settings within a month so that the variations in microscope light source and sensitivity were negligible (35). Calibration was thus not necessary since only relative signals of NADH and Fp in arbitrary units (a.u.) are reported.
Drugs
Chemical agents were purchased from Sigma-Aldrich (Allentown, PA, USA), except MitoSOX red was purchased from Thermo-Fisher Scientific. The chemical agents were reconstituted in dimethyl sulfoxide (DMSO), aliquoted, and stored at either −20 or −80 ℃.
Redox plasticity measurements
Redox plasticity measurements were achieved by sequentially adding mitochondrial metabolic perturbation drugs trifluoromethoxy carbonylcyanide phenylhydrazone (FCCP) and a mixture of rotenone (ROT) and antimycin A (AA) (ROTAA). Drugs were added to cell dishes at the following concentrations: FCCP 0.5 µM, ROT 1 µM, AA 1.25 µg/mL. Images were taken approximately 3–5 minutes post-addition of each drug.
ROS measurements
Cytoplasmic ROS measurements were acquired by adding dihydroethidium (DHE; 2 µM final) to cells and incubating at 37 ℃ protected from light for 40 minutes. Mitochondrial ROS measurements were acquired by adding MitoSOX (2.5 µM final) to cells and incubating at 37 ℃ protected from light for 10 minutes. In both cases, dishes were then rinsed once with DPBS+ and liquid was replaced with 1 mL LCIS+ for imaging using the Zeiss Axio Observer 7 microscope with the following bandpass filters: DHE Ex 540–570 nm, Em 580–610 nm; MitoSOX Ex 370–400 nm, Em 580–610 nm. Both cytoplasmic and mitochondrial ROS levels were measured under control, FCCP treatment, and FCCP + ROTAA treatment. Cytoplasmic and mitochondrial ROS measurements were taken for control and FCCP (0.5 µM)-treated dishes after 3–5 minutes of treatment, then incubated with either DHE or MitoSOX, respectively. Cytoplasmic ROS measurements under FCCP + ROTAA treatment were collected immediately after the plasticity measurements (1 µM FCCP added then 3 dishes imaged taking ~15 minutes, then ROTAA added sequentially to dish and 3 dishes imaged for ~15 minutes in total) when DHE was added to FCCP + ROTAA treated dishes and incubated before imaging the tracer.
Data analysis and statistics
Files obtained via fluorescence microscopy were separated into single channels using ImageJ software. The split NADH, Fp, and ROS images were used in a custom MATLAB program that operates through a series of background flattening and signal thresholding at the signal-to-noise ratio [(signal − background)/background standard deviation (SD)] greater than 7.5 to determine NADH, Fp, and ROS intensities (10). Images of the redox ratio Fp/(NADH + Fp) were created pixel-by-pixel from the net intensity images of NADH and Fp. Means were obtained by averaging 3–5 fields of view (FOV) per dish, and then averaging across dishes. For separate analysis of early or late passage measurements, independent replicates n=6 for each group. For analysis pooling both early and late passage data, independent replicates n=12 for each group. The MitoSOX measurement for one MDA-MB-231 dish (early passage under basal condition) was regarded as an outlier with signal deviation 1.5 times the interquartile range (IQR) below the first quartile or above the third quartile and removed from further analysis. Bar graphs are displayed as the calculated mean ± SD. One- or two-way analysis of variance (ANOVA) analysis followed by post-hoc Tukey or Bonferroni tests (correction for multiple comparison) were performed to make comparisons between >2 groups. For comparison of the two groups, unpaired t-tests with unequal variance were utilized. All statistical tests were performed using PRISM software (v8-10; GraphPad, Boston, MA, USA). Significant differences are graphically displayed as: *, P<0.05; **, P<0.01; ***, P<0.001; and ****, P<0.0001.
Results
No significant morphological changes between early and late passage cells in both lines
First, we did not visually observe any significant morphology changes between the early and late passage cells for MDA-MB-231 and HCC1806 cells (Figure 1), after having propagating MDA-MB-231 cells until passage 70 (P70) and HCC1806 cells to P86.
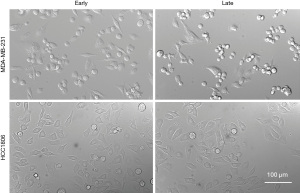
Passage dependence of NADH redox status under basal condition and metabolic perturbations
As indicated in Figure 2A, ORI found no significant differences in the basal levels of Fp, NADH, or redox ratios between the early and late passage MDA-MB-231 cells. Both early and late passage MDA-MB-231 cells responded similarly to metabolic perturbations induced by sequential FCCP and ROTAA treatments. FCCP uncoupling reduced NADH and increased redox ratio while ROTAA inhibition of respiration increased NADH and decreased redox ratio. Under FCCP treatment, there were no significant Fp or NADH differences between early and late passage cells but the redox ratio was significantly higher in the late passage cells. After adding ROTAA, the redox ratio remained ~25% higher and NADH was ~25% lower in the late passage cells than in the early passage cells. In other words, early passage cells show a stronger response to ROTAA treatment than late passage cells, specifically a larger increase in NADH and a larger redox ratio drop after ROTAA was added. Late passage cells demonstrate a nearly four-fold increase in NADH after ROTAA was added, as to where early passage cells demonstrate a five-fold increase in NADH, making early and late passage cell NADH values different from each other after addition of ROTAA.
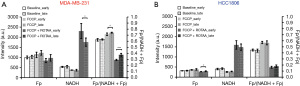
Likewise, early passage and late passage HCC1806 cells exhibited no change in the baseline NADH redox status. All metabolically-perturbed levels of Fp, NADH, and redox ratio showed no significant passage-dependent differences except the Fp under FCCP + ROTAA treatment (Figure 2B). Fp was significantly higher in later passage cells by 8.5% than early passage cells.
Thus, both TNBC cell lines showed similar redox baselines between early and late passage cells, with no passage-dependent changes in any redox indices. Only MDA-MB-231 cells exhibited increase of redox ratio under FCCP treatment. Some redox differences appeared under metabolic perturbations of FCCP + ROTAA for both lines, with HCC1806 showing a trend of redox ratio increase and MDA-MB-231 showing a more significant increase of redox ratio and a more oxidized redox status in late passage cells.
Passage dependence of redox plasticity
To evaluate passage dependence of the reserve pool sizes of redox indices, we conducted optical redox plasticity measurements. The plasticity of cellular Fp, NADH, and redox ratio were obtained by taking the difference of each index between the FCCP and FCCP + ROTAA conditions. For example, the difference of NADH between FCCP and FCCP + ROTAA conditions is denoted as NADH plasticity (ΔNADH), reflecting the cellular NADH pool size and/or regenerating capacity. Likewise, we obtained Fp plasticity (ΔFp) and redox ratio plasticity (Δratio). The late passage MDA-MB-231 cells had a decrease in both ΔNADH and Δratio (Figure 3A). Between early and late passage cell lines, the NADH plasticity differs by 37% and the redox ratio plasticity differs by 14.5%, while Fp plasticity (ΔFp) shows no change. For HCC1806, early and late passage cells are observed to have similar redox plasticity (ΔFp, ΔNADH, and Δratio) despite a trend of decrease of ΔNADH and Δratio in late passage cells (P>0.05) (Figure 3B). Thus, late passage MDA-MB-231 cells exhibited reduced the plasticity of NADH and redox ratio compared to early passage cells, while HCC1806 cells showed no significant differences.
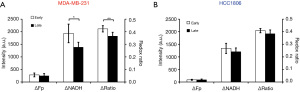
Passage dependence of ROS levels
Furthermore, we investigated the passage-dependence of cellular ROS level. Early and late passage MDA-MB-231 cells have the same amount of cytoplasmic ROS at baseline based on the DHE fluorescence measurement (Figure 4A). Furthermore, both passage groups had significant cytoplasmic ROS increases (>100%) responding to FCCP, and even more significant increases (~300%) in cytoplasmic ROS in response to combination of FCCP and ROTAA. However, there are no significant differences between early and late passage groups under each condition. Similar to MDA-MB-231 cells as shown in Figure 4B, HCC1806 cells exhibited no difference in cytoplasmic ROS between early and late passage cells under baseline condition, FCCP treatment, or combination of FCCP + ROTAA treatment. FCCP + ROTAA doubled or more than doubled the cytoplasmic ROS level compared to control and FCCP groups in HCC1806 cells. However, FCCP induced no significant cytoplasmic ROS increase in HCC1806 cells which differs from the MDA-MB-231 line.
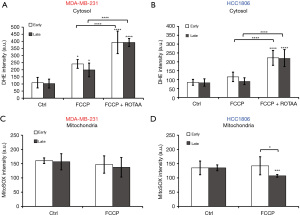
With MitoSOX staining, we measured the passage dependence of mitochondrial ROS at basal condition or in response to FCCP treatment. We observed the same amount of mitochondrial ROS in both early and late passage cells at baseline and FCCP treatment induced no change in MDA-MB-231 cells (Figure 4C). Early passage HCC1806 cells demonstrated no change in mitochondrial ROS in response to FCCP treatment, but late passage cells demonstrated a significant decrease in mitochondrial ROS in response to FCCP treatment (Figure 4D). There is a significant 28% difference between the mitochondrial ROS levels of early vs. late passage cells under FCCP treatment, although there was no difference in initial baseline values between passages.
Thus, we observed that MDA-MB-231 cells do not show significant passage-dependent differences in ROS levels under both baseline and metabolic perturbations. HCC1806 cells show no passage-dependent difference in cytosolic ROS level under both baseline and metabolic perturbations; late passage cells exhibit significantly lower mitochondrial ROS generation in response to FCCP.
Redox and ROS differences between TNBC cell lines
Lastly, we compare the differences in NADH redox indices and ROS levels between the more invasive/metastatic MDA-MB-231 and the less invasive/metastatic HCC1806 cells. Figure 5 shows the redox indices (including Fp, NADH, redox ratios, and redox plasticity parameters) by pooling the early and late passage data together for each specific cell line. Passage-dependent comparisons are shown in Figures 6,7 for early and late passage cells, respectively. Regardless whether using passage-dependent data or pooled data, the comparisons of redox indices between the two cell lines yield similar results. Under the basal (treatment naïve) condition, MDA-MB-231 cells have similar NADH, but significantly higher Fp and redox ratio compared to HCC1806 cells (Figures 5,6,7A,7B,7C), consistent with the results of our previous studies (13,32) that linked more oxidized NADH redox status to higher invasiveness of breast cancer cells in vitro. Such relationship is maintained under the metabolic perturbations by FCCP and FCCP + ROTAA. Moreover, MDA-MB-231 cells exhibit significantly higher plasticity in Fp than HCC1806 cells regardless pooling passage-dependent data or not (Figures 5-7). In contrast, NADH plasticity was significantly higher in MDA-MB-231 cells than HCC1806 cells only in the pooled data and data of the early-passage cells. For the late-passage cells, no significant NADH plasticity difference was observed between the two cell models (Figure 7D). There was no difference in the redox ratio plasticity between the two lines.
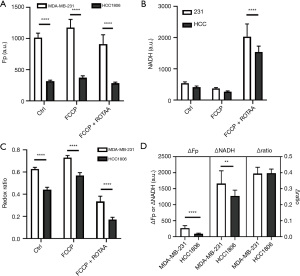
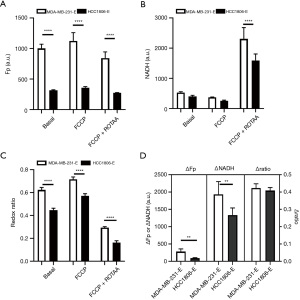
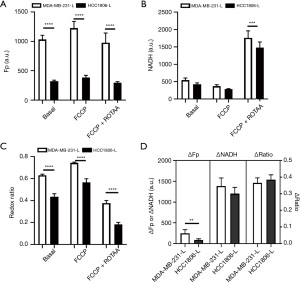
Figure 8 compares the intracellular ROS levels between the two cell lines by pooling data from early and late passages since largely there are no significant differences in ROS levels between early and late passages (Figure 4). As shown in Figure 8A, the more oxidized NADH redox status is associated with an observed higher cytoplasmic ROS level in MDA-MB-231 compared to HCC1806 under both the basal condition and metabolic perturbations by FCCP or FCCP + ROTAA. However, the mitochondrial ROS levels appear to be similar between the two cell lines under the control and FCCP treatment conditions (P>0.05) (Figure 8B). Our data indicate that the more aggressive (invasive/metastatic) MDA-MB-231 cells consistently exhibited a more oxidized NADH redox status and higher cytoplasmic ROS levels compared to HCC1806 cells, under both basal and perturbed conditions.
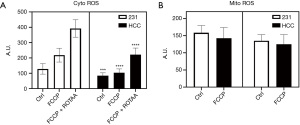
Discussion
Key findings and explanations
In this study, we found no significant passage-dependent differences in the baseline NADH redox status (NADH, Fp, and redox ratio) for two TNBC cell lines, MDA-MB-231 and HCC1806 (Figure 1). However, under metabolic perturbation of mitochondrial electron transport chain activity by an uncoupler FCCP or a complex I and III inhibitor ROTAA, passage-dependent differences in redox indices were identified in MDA-MB-231 cells, with the late passage exhibiting lower NADH and becoming more oxidized with higher redox ratios. A slight increase of Fp was also found for HCC1806 late passage cells under the treatment of FCCP + ROTAA. Furthermore, both the NADH plasticity and redox ratio plasticity were significantly reduced in late passage MDA-MB-231 cells compared to early passage cells (Figure 3). In contrast, no significant redox plasticity differences were found in HCC1806 cells under either baseline or metabolic perturbation conditions. Neither cell line exhibited a significant difference in cytoplasmic ROS level between early and late passages under baseline, FCCP perturbed, or FCCP + ROTAA perturbed conditions (Figure 4). As for mitochondrial ROS level, both cell lines showed no passage-dependent differences under baseline condition. Under FCCP perturbed condition, MDA-MB-231 showed no dependence on passage number whereas HCC1806 showed a significant decrease of ROS level in late passage cells.
We also found the more aggressive/invasive MDA-MB-231 line had a more oxidized NADH redox status (higher Fp and redox ratios), higher Fp and NADH plasticity, and higher cytoplasmic ROS level than the less aggressive/invasive HCC1806 line (Figures 5-8). Such relationship held largely (with the exception of NADH plasticity for late passage cells and the redox ratio plasticity in pooled or passage-separated analyses) regardless of metabolic perturbation conditions and cell passage (either early or late passage or pooled together for analysis) implying its generality.
Comparison to previous studies
Although cancer cell environment and biology may change with passaging in general, it remains unclear whether and how the metabolic/redox features of cancer cells change with passage number in vitro. Passage or senescence-dependence of ROS/oxidative stress have been reported in non-cancer cell studies (36-38), but not reported in mature cancer cells. Cancer cells have been considered immortal and more robust than non-cancer cells, and they are expected to be better at maintaining cell phenotypes after repeated culture propagation. Consistently, in these two TNBC cell lines, we have found no significant changes in either morphology or baseline NADH redox status and cellular ROS levels. Some passage-dependent drifts in the redox/ROS profiles were found only under metabolic perturbations, appearing before significant morphology changes.
Previously we studied the relationship between NADH redox status and cytoplasmic ROS levels in MDA-MB-231 cells without considering passage dependence (23). A higher cytoplasmic ROS level can be associated with either more oxidized or more reduced redox status. In this study, we found similar NADH redox baseline/plasticity responses and cytoplasmic ROS responses in two TNBC cell lines HCC1806 and MDA-MB-231. Under the metabolic perturbation of FCCP followed by ROTAA, compared to baseline, we found that the redox ratio was increased significantly (more oxidation) by FCCP, which uncouples the electron transport chain from oxidative phosphorylation and enhances the consumption of reducing equivalency and oxygen, and it was decreased significantly (more reduction) by FCCP + ROTAA, which inhibits the electron transport chain at complex I and III, as expected from previous studies (23). However, under both treatment conditions, the cytoplasmic ROS levels were increased significantly with an even higher ROS level for FCCP + ROTAA in MDA-MB-231 cells. In HCC1806 cells, cytoplasmic ROS levels did not change with FCCP treatment but increased significantly with FCCP + ROTAA treatment. Thus, these results confirmed the previous observation that the change of intracellular ROS levels may not correlate with the change of NADH redox status detected by ORI. Furthermore, the MitoSOX responses are inconsistent with the previous study (23), which showed that FCCP decreased mitochondrial ROS in MDA-MB-231 cells and increased it in HCC1806; instead mitochondrial ROS in both cell lines failed to respond to FCCP stimulation significantly except the late passage HCC1806 showing a decrease. It remains unclear what may have caused such apparent discrepancies, and more investigations are needed in the future.
Our results comparing the differences between the two TNBC cell models are consistent with a previous study that indicates a higher redox ratio under the basal condition and a higher Fp plasticity in the more invasive MDA-MB-231 line compared to the less invasive HCC1806 line (39). There is also a significant correlation of basal ORR and invasiveness among four TNBC cell lines (32). Furthermore, MDA-MB-231 cells have been found to have a more oxidized ORI-detected redox status than the less invasive/aggressive estrogen-receptor positive MCF-7 cells under both baseline and various nutritional conditions including starvation and supplementation of glucose and glutamine (13). A review paper has also pointed out a correlation between the ORR and the rank orders of invasive potential in multiple breast cancer cell lines (40). All these results support the hypothesis that more oxidized NADH redox status is an indicator of intrinsic invasive nature of TNBC cells. It is interesting to observe that the more oxidized NADH redox status is associated with a higher cytoplasmic ROS instead of mitochondrial ROS. The underlying mechanism remains to be investigated. It also remains to be tested whether the association of more oxidized NADH redox and higher cytoplasmic ROS levels is a general feature of the treatment-naïve TNBC cells.
Strengths and limitations
The strengths of this research include: (I) utilizing endogenous and noninvasive fluorescent indicators of cellular redox metabolism; (II) correlating the redox/ROS differences between two representative human TNBC lines to identify their associations with invasive potential; and (III) investigating the temporal change of redox/ROS status along with multiple passages of cell culturing. The study is limited to in vitro models only.
Implications and actions needed
The basic knowledge of the evolution of metabolic/redox phenotypes during cancer cell culturing obtained by this study is valuable for more accurately defining the effect of drug treatment on metabolic/redox phenotypes of cancer cells in vitro. The NADH redox/ROS status of two representative human TNBC lines and their associations with the rank order of invasive/metastatic potential have been confirmed to be largely insensitive to the passage number, supporting redox/ROS indicators as potential biomarkers for TNBC aggressiveness. The in vitro results were obtained under normoxia and need to be tested under hypoxia, the presence of which in tumors is known to drive malignancy and progression. If validated, they can be combined with future studies of animal models and human tissues ex vivo to reach general conclusions about the significance and value of these redox/ROS biomarkers for TNBC prognosis.
Conclusions
In summary, we have investigated the passage-dependence of the intracellular redox/ROS indices from two representative TNBC cell lines with different levels of invasive/metastatic potential. We showed that the potential prognostic biomarkers based on redox/ROS measurements can differentiate these two lines without significant dependence on the passage numbers, supporting the general validity of the redox/ROS biomarkers representing intrinsic nature of TNBC aggressiveness. In the future, we can test this hypothesis in more TNBC cells under the hypoxia condition and mouse models and clinical specimens and even expand the study to other breast cancer molecular subtypes.
Acknowledgments
We thank the Cell and Developmental Biology (CDB) Microscopy Core, Perelman School of Medicine, University of Pennsylvania for technical assistance
Funding: This work was partially supported by
Footnote
Reporting Checklist: The authors have completed the MDAR reporting checklist Available at https://tbcr.amegroups.org/article/view/10.21037/tbcr-24-36/rc
Data Sharing Statement: Available at https://tbcr.amegroups.org/article/view/10.21037/tbcr-24-36/dss
Peer Review File: Available at https://tbcr.amegroups.org/article/view/10.21037/tbcr-24-36/prf
Conflicts of Interest: All authors have completed the ICMJE uniform disclosure form (available at https://tbcr.amegroups.org/article/view/10.21037/tbcr-24-36/coif). L.Z.L. serves as an unpaid editorial board member of Translational Breast Cancer Research from May 2023 to April 2025. L.Z.L. was supported by the Perelman School of Medicine Bridge Fund at the University of Pennsylvania and NIH Grants R01-CA191207 and R01-CA277037. H.N.X. received partial support from the McCabe Pilot Award at the University of Pennsylvania and NIH Grants R01-CA191207 for this work. The other author has no conflicts of interest to declare.
Ethical Statement: The authors are accountable for all aspects of the work in ensuring that questions related to the accuracy or integrity of any part of the work are appropriately investigated and resolved.
Open Access Statement: This is an Open Access article distributed in accordance with the Creative Commons Attribution-NonCommercial-NoDerivs 4.0 International License (CC BY-NC-ND 4.0), which permits the non-commercial replication and distribution of the article with the strict proviso that no changes or edits are made and the original work is properly cited (including links to both the formal publication through the relevant DOI and the license). See: https://creativecommons.org/licenses/by-nc-nd/4.0/.
References
- Lehninger AL, Nelson DL, Cox MM. Principles of Biochemistry. 2nd ed. New York: Worth Publishers; 1993.
- Goodman RP, Calvo SE, Mootha VK. Spatiotemporal compartmentalization of hepatic NADH and NADPH metabolism. J Biol Chem 2018;293:7508-16. [Crossref] [PubMed]
- Li LZ, Xu HN, Ranji M, et al. Mitochondrial redox imaging for cancer diagnostic and therapeutic studies. J Innov Opt Health Sci 2009;2:325-41. [Crossref] [PubMed]
- Quinn KP, Sridharan GV, Hayden RS, et al. Quantitative metabolic imaging using endogenous fluorescence to detect stem cell differentiation. Sci Rep 2013;3:3432. [Crossref] [PubMed]
- Varone A, Xylas J, Quinn KP, et al. Endogenous two-photon fluorescence imaging elucidates metabolic changes related to enhanced glycolysis and glutamine consumption in precancerous epithelial tissues. Cancer Res 2014;74:3067-75. [Crossref] [PubMed]
- Chance B, Schoener B, Oshino R, et al. Oxidation-reduction ratio studies of mitochondria in freeze-trapped samples. NADH and flavoprotein fluorescence signals. J Biol Chem 1979;254:4764-71.
- Hou J, Wright HJ, Chan N, et al. Correlating two-photon excited fluorescence imaging of breast cancer cellular redox state with seahorse flux analysis of normalized cellular oxygen consumption. J Biomed Opt 2016;21:60503. [Crossref] [PubMed]
- Gu Y, Qian Z, Chen J, et al. High-resolution three-dimensional scanning optical image system for intrinsic and extrinsic contrast agents in tissue. Rev Sci Instrum 2002;73:172-8.
- Kolenc OI, Quinn KP. Evaluating Cell Metabolism Through Autofluorescence Imaging of NAD(P)H and FAD. Antioxid Redox Signal 2019;30:875-89. [Crossref] [PubMed]
- Wen Y, Xu HN, Privette Vinnedge L, et al. Optical Redox Imaging Detects the Effects of DEK Oncogene Knockdown on the Redox State of MDA-MB-231 Breast Cancer Cells. Mol Imaging Biol 2019;21:410-6. [Crossref] [PubMed]
- Xu HN, Feng M, Moon L, et al. Redox imaging of the p53-dependent mitochondrial redox state in colon cancer ex vivo. J Innov Opt Health Sci 2013;6:1350016. [Crossref] [PubMed]
- Xu HN, Nioka S, Glickson JD, et al. Quantitative mitochondrial redox imaging of breast cancer metastatic potential. J Biomed Opt 2010;15:036010. [Crossref] [PubMed]
- Sun N, Xu HN, Luo Q, et al. Potential Indexing of the Invasiveness of Breast Cancer Cells by Mitochondrial Redox Ratios. Adv Exp Med Biol 2016;923:121-7. [Crossref] [PubMed]
- Li LZ, Zhou R, Xu HN, et al. Quantitative magnetic resonance and optical imaging biomarkers of melanoma metastatic potential. Proc Natl Acad Sci U S A 2009;106:6608-13. [Crossref] [PubMed]
- Li LZ. Imaging mitochondrial redox potential and its possible link to tumor metastatic potential. J Bioenerg Biomembr 2012;44:645-53. [Crossref] [PubMed]
- Peng A, Xu HN, Moon L, et al. Quantitative Optical Redox Imaging of Melanoma Xenografts with Different Metastatic Potentials. Cancers (Basel) 2024;16:1669. [Crossref] [PubMed]
- Xu HN, Mir T, Lee SC, et al. Mapping the Redox State of CHOP-Treated Non-Hodgkin’s Lymphoma Xenografts in Mice. In: Van Huffel S, Naulaers G, Caicedo A, et al. editors. Oxygen Transport to Tissue XXXV. New York: Springer; 2013:243-9.
- Xu HN, Zhao H, Mir TA, et al. Chop therapy induced mitochondrial redox state alteration in non-Hodgkin’s lymphoma xenografts. J Innov Opt Health Sci 2013;6:1350011. [Crossref] [PubMed]
- Xu HN, Feng M, Nath K, et al. Optical Redox Imaging of Lonidamine Treatment Response of Melanoma Cells and Xenografts. Mol Imaging Biol 2019;21:426-35. [Crossref] [PubMed]
- Walsh AJ, Cook RS, Manning HC, et al. Optical metabolic imaging identifies glycolytic levels, subtypes, and early-treatment response in breast cancer. Cancer Res 2013;73:6164-74. [Crossref] [PubMed]
- Walsh A, Cook RS, Rexer B, et al. Optical imaging of metabolism in HER2 overexpressing breast cancer cells. Biomed Opt Express 2012;3:75-85. [Crossref] [PubMed]
- Podsednik A, Jiang J, Jacob A, et al. Optical Redox Imaging of Treatment Responses to Nampt Inhibition and Combination Therapy in Triple-Negative Breast Cancer Cells. Int J Mol Sci 2021;22:5563. [Crossref] [PubMed]
- Podsednik A, Jacob A, Li LZ, et al. Relationship between Optical Redox Status and Reactive Oxygen Species in Cancer Cells. React Oxyg Species (Apex) 2020;9:95-108.
- Esquenet M, Swinnen JV, Heyns W, et al. LNCaP prostatic adenocarcinoma cells derived from low and high passage numbers display divergent responses not only to androgens but also to retinoids. J Steroid Biochem Mol Biol 1997;62:391-9. [Crossref] [PubMed]
- Briske-Anderson MJ, Finley JW, Newman SM. The influence of culture time and passage number on the morphological and physiological development of Caco-2 cells. Proc Soc Exp Biol Med 1997;214:248-57. [Crossref] [PubMed]
- Chang-Liu CM, Woloschak GE. Effect of passage number on cellular response to DNA-damaging agents: cell survival and gene expression. Cancer Lett 1997;113:77-86. [Crossref] [PubMed]
- Yu H, Cook TJ, Sinko PJ. Evidence for diminished functional expression of intestinal transporters in Caco-2 cell monolayers at high passages. Pharm Res 1997;14:757-62. [Crossref] [PubMed]
- Sundareshan P, Hendrix MJ. Growth, morphologic, and invasive characteristics of early and late passages of a human endometrial carcinoma cell line (RL95-2). In Vitro Cell Dev Biol 1992;28A:544-52. [Crossref] [PubMed]
- Mouriaux F, Zaniolo K, Bergeron MA, et al. Effects of Long-term Serial Passaging on the Characteristics and Properties of Cell Lines Derived From Uveal Melanoma Primary Tumors. Invest Ophthalmol Vis Sci 2016;57:5288-301. [Crossref] [PubMed]
- Kruczynski A, Kiss R. Evidence of a direct relationship between the increase in the in vitro passage number of human non-small-cell-lung cancer primocultures and their chemosensitivity. Anticancer Res 1993;13:507-13.
- Lehmann BD, Bauer JA, Chen X, et al. Identification of human triple-negative breast cancer subtypes and preclinical models for selection of targeted therapies. J Clin Invest 2011;121:2750-67. [Crossref] [PubMed]
- Feng M, Xu HN, Jiang J, et al. Potential Biomarker for Triple-Negative Breast Cancer Invasiveness by Optical Redox Imaging. Adv Exp Med Biol 2021;1269:247-51. [Crossref] [PubMed]
- Aslan M, Hsu EC, Liu S, et al. Quantifying the invasion and migration ability of cancer cells with a 3D Matrigel drop invasion assay. Biol Methods Protoc 2021;6:bpab014. [Crossref] [PubMed]
- Cailleau R, Young R, Olivé M, et al. Breast tumor cell lines from pleural effusions. J Natl Cancer Inst 1974;53:661-74. [Crossref] [PubMed]
- Jacob A, Xu HN, Stout AL, et al. Subcellular analysis of nuclear and cytoplasmic redox indices differentiates breast cancer cell subtypes better than nuclear-to-cytoplasmic area ratio. J Biomed Opt 2022;27:086001. [Crossref] [PubMed]
- Bocchino M, Agnese S, Fagone E, et al. Reactive oxygen species are required for maintenance and differentiation of primary lung fibroblasts in idiopathic pulmonary fibrosis. PLoS One 2010;5:e14003. [Crossref] [PubMed]
- Crowder SW, Horton LW, Lee SH, et al. Passage-dependent cancerous transformation of human mesenchymal stem cells under carcinogenic hypoxia. FASEB J 2013;27:2788-98. [Crossref] [PubMed]
- Prieto I, Zambrano A, Laso J, et al. Early induction of senescence and immortalization in PGC-1α-deficient mouse embryonic fibroblasts. Free Radic Biol Med 2019;138:23-32. [Crossref] [PubMed]
- Jiang J, Feng M, Jacob A, et al. Optical Redox Imaging Differentiates Triple-Negative Breast Cancer Subtypes. Adv Exp Med Biol 2021;1269:253-8. [Crossref] [PubMed]
- Li LZ, Sun N. Autofluorescence perspective of cancer diagnostics. In: Ghukasyan V, Heikal AA. editors. Natural Biomarkers for Cellular Metabolism: Biology, Techniques, and Applications. New York: CRC Press; 2014:273-97.
Cite this article as: Podsednik A, Xu HN, Li LZ. Passage dependence of NADH redox status and reactive oxygen species level in vitro in triple-negative breast cancer cell lines with different invasiveness. Transl Breast Cancer Res 2024;5:27.